The mission of Explorer 1 was assembled in a hurry. The US was trailing the USSR in space exploration, and the first attempted launch of its official entry, the Vanguard, ended in flames on the launching pad, and a launch vehicle was therefore improvised from available components, a back-up plan devised earlier by Von Braun [1964; Pickering, 1963]. The resulting orbit was rather non-circular and rose to an apogee of 2500 km, deep inside the radiation belt. Explorer 1 carried a radiation detector, a Geiger counter provided by Van Allen's team at the University of Iowa (Figure 1), which also included Carl McIlwain, Ernie Ray and George Ludwig [Van Allen, 1981, 1983a]. The counter was meant to measure the overall cosmic ray intensity, which by Stoermer's theory [see BH-1] was expected to increase with magnetic latitude, and its predicted counting rate was about 30 counts per second. The experimental package was a modified version of one designed for a later launch in the Vanguard series, and included a tape recorder designed to store the data for retransmission when the spacecraft passed over tracking stations. However, it was decided to provide this feature only on later missions and to omit it from Explorer 1, so that stations were only able to collect a few minutes' worth of data whenever Explorer 1 passed within range.
On passes below 600 km the counting rate was nominal. Near apogee, however, no counts at all were detected, and on one pass, with the spacecraft around 1200 km and rising, counts were received but suddenly stopped [Van Allen, 1981, Fig.8]. Actually, much of this was only noted later: what was mainly noted was that sometimes the counter on the spacecraft operated normally and at other times it seemed dead. McIlwain showed experimentally that very high particle fluxes would overwhelm the counter and produce zero counts, and there is little doubt that further analysis of Explorer 1 data would probably have led to the discovery of the radiation belt. Before that could happen, however, much less ambiguous data were obtained from another experiment.
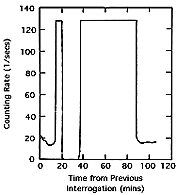
Figure 2. Counting rate of
Explorer 3 during a pass
through the radiation belt.
The highest rate was encoun-
tered during the segment
of zero counts.
Explorer 2 failed to orbit, but Explorer 3 was successful. Unlike Explorer 1, it carried a tape recorder, and its continuous record of data (Figure 2) made clear what was happening. At low altitudes, only cosmic rays were detected; then as the satellite rose the recorded counting rate increased up to the highest it could record, and it stayed pegged there for a while. At a still higher altitude it abruptly fell to zero, and during descent the same transitions occurred in reverse order. The periods of zero counts near apogee clearly marked not the absence of radiation but a very high radiation flux: the Geiger counter was discharged so frequently that it did not recover between pulses and its output signals decreased until they no longer triggered the counting circuit.
That was how the radiation belt was discovered [Van Allen et al., 1958; Van Allen, 1981]. Ernie Ray's comment was: "My God, space is radioactive!" [Hess, 1968]. The identity of the particles was quite uncertain. Auroral electrons had been observed in near-Earth space, but they lacked the energy to penetrate the counter walls. They could trigger a count by means of secondary X-rays, with a probability of the order of 10-5 [Frank, 1962], an explanation which had been previously used to explain rocket observations in the auroral zone [Van Allen, 1995]. Such an interpretation would have implied a huge flux of electrons, of order 100 million per cm-sq. sec, a figure which proponents of manned space flight viewed with justified alarm. Sputnik 3, launched May 12 to an apogee of 1880 km, carried scintillation detectors and confirmed the existence of Van Allen's belt, but it did not resolve the identity of the particles, and neither did Explorer 4, discussed in the next section [Van Allen et al., 1959]. As noted further below, the particles of the intense innermost part of the belt were soon accounted for. It was later pointed out by Dessler [1960; also Dessler and Vestine, 1960] that the extremely high fluxes, implied by the X-ray interpretation, would have modified the Earth's field above and beyond the changes actually observed. However, the uncertainty about the outer belt persisted until the work of Davis and Williamson [1963, 1966] and Frank's electron measurements with OGO 3 [Frank, 1967].
In conclusion, it should be stressed here that the above is a very abbreviated summary of a complex period of discovery, and a much more detailed picture is available from Van Allen [1983a].